- Abstract:
- 1 Introduction▲
- 2 Nonlinear failure ...▲
- 3 Upper bound limit ...▲
- 4 Comparisons and an...▲
- 5 Analyses of parame...▲
- 6 Conclusions▲
- References
- Figure
- Figure 1 Nonlinear MC failure criterion
- Figure 2 Failure mechanism of 2-layer soil above a strip anchor plate
- Figure 3 Failure mechanism of single-layer soil above a shallow strip anchor plate
- Figure 4 Comparison of ultimate pullout force and failure mechanism of single-layer soil above strip anchor plate under linear MC criterion:
- Figure 5 Comparison of ultimate pullout force and failure mechanism of single-layer soil above strip anchor plate under nonlinear MC criterion:
- Figure 6 Variation curve of ultimate pullout force versus m1
- Figure 7 Variation curve of ultimate pullout force versus ratio of γ
- Figure 8 Variation curve of ultimate pullout force versus ratio of C0
- Figure 9 Variation curve of ultimate pullout force versus friction angle of soil mass
- Figure 10 Variation curve of ultimate pullout force versus q
- Figure 11 Variation curve of ultimate pullout force versus ratio of σt2/σt1
- Figure 12 Variation curve of ultimate pullout force versus depth under different conditions of unit weight of soil:
- Figure 13 Variation curve of ultimate pullout force versus depth under different conditions of uniaxial tensile strength:
- Figure 14 Variation curve of ultimate pullout force versus depth under different conditions of initial cohesion:
- Figure 15 Influence of nonlinear coefficient
- Figure 16 Influence of unit weight of soil
- Figure 17 Influence of initial cohesion
- Figure 18 Influence of friction angle of soil mass
- Figure 19 Influence of surface overload
- Figure 20 Influence of uniaxial tensile strength
- Figure 21 Influence of depth under different conditions of unit weight of soil:
- Figure 22 Influence of depth under different conditions of uniaxial tensile strength of soil mass:
- Figure 23 Influence of depth under different conditions of initial cohesion:
J. Cent. South Univ. (2018) 25: 2802-2818
DOI: https://doi.org/10.1007/s11771-018-3954-x
Variation analysis of ultimate pullout capacity of shallow horizontal strip anchor plate with 2-layer overlying soil based on nonlinear M-C failure criterion
ZHAO Lian-heng(赵炼恒)1, 2, TAN Yi-gao(谭亦高)1, NIE Zhi-hong(聂志红)1,YANG Xin-ping(杨新平)1, HU Shi-hong(胡世红)1
1. School of Civil Engineering, Central South University, Changsha 410075, China;
2. Key Laboratory of Heavy-haul Railway Engineering Structure of Ministry of Education,Changsha 410075, China
Central South University Press and Springer-Verlag GmbH Germany, part of Springer Nature 2018
Abstract:
Based on the nonlinear Mohr-Coulomb failure criterion and an associated flow rule, a kinematic admissible velocity field of failure mechanism of the 2-layer soil above a shallow horizontal strip anchor plate is constructed. The ultimate pull-out force and its corresponding failure mechanism through the upper bound limit analysis according to a variation principle are deduced. When the 2-layer overlying soil is degraded into single-layer soil, the model of ultimate pullout force could also be degraded into the model of single-layer soil. And the comparison between results of single-layer soil variation method and those calculated by rigid limit analysis method proves the correctness of our method. Based on that, the influence of changes of geotechnical parameters on ultimate pullout forces and failure mechanism of a shallow horizontal strip anchor with the 2-layer soil above are analyzed. The results show that the ultimate pull-out force and failure mechanism of a shallow horizontal strip anchor with the 2-layer soil above are affected by the nonlinear geotechnical parameters greatly. Thus, it is very important to obtain the accurate geotechnical parameters of 2-layer soil for the evaluation of the ultimate pullout capacity of the anchor plate.
Key words:
Cite this article as:
ZHAO Lian-heng, TAN Yi-gao, NIE Zhi-hong, YANG Xin-ping, HU Shi-hong. Variation analysis of ultimate pullout capacity of shallow horizontal strip anchor plate with 2-layer overlying soil based on nonlinear M-C failure criterion [J]. Journal of Central South University, 2018, 25(11): 2802-2818.
DOI:https://dx.doi.org/https://doi.org/10.1007/s11771-018-3954-x1 Introduction
The uplift anchor plate foundation is widely used in offshore platforms, transmission towers, television towers, towering structures and a variety of structure foundations under uplifting load. The anchor plates are mainly divided into strip anchor plate, rectangular anchor plate and circular anchor plate according to their shapes and could be divided into deep buried anchor plate and shallow anchor plate according to the buried depth. As a basic form to provide the pull-out force, the anchor plate is widely used in practical engineering, and its bearing capacity, especially the ultimate pullout capacity, serves as focus of the research of uplift-resistant foundation. At present, there are a large number of studies for the ultimate pullout capacity of anchor plates. The main methods are model test method [1–13], limit equilibrium method [1, 5, 8, 13–15], limit analysis method [3, 13, 16–25] and numerical simulation method [10–12, 15, 16, 26–31].
At present, it is assumed that the soil material overlying anchor plate obeys the linear Mohr- Coulomb failure criterion (i.e., the M-C criterion). However, a large number of studies have shown that almost all failure criteria of the geomaterials have non-linear characteristics and the present commonly used linear M-C failure criterion is only a special case [31–37]. But based on the nonlinear failure criterion of geomaterials, the influence of the ultimate pull-out force of shallow anchor plate needs further studies. ZHAO et al [18] analyzed the ultimate pull-out force of the strip anchor plate, the circular anchor plate and the rectangular anchor plate under the nonlinear MC criterion. These results show that the nonlinear parameters have an important effect on the ultimate pullout force of the anchor plate and the linear simplification of nonlinear geomaterials is not conducive to the correct evaluation of the bearing capacity of the uplift-resistant foundation. Therefore, it is necessary to consider the nonlinear characteristics of failure criterion of geomaterials for the correct evaluation of pullout capacity of the anchor plate.
The studies in Ref. [18] are based on the assumption of simple failure mechanism because of the nonlinear characteristics of shear strength of the geomaterials, but the assumption is not in line with the engineering practice. Taking this deficiency into account, WANG et al [38] studied the ultimate pull-out force of a 2-dimensional strip anchor plate based on the variation method in the framework of the upper bound theory. The study does not presume the form of the failure mechanism, but derives the failure mechanism of 2-dimensional curves for the 2-dimensional strip anchor by strict theoretical derivation, with which the calculation result is obviously better than that of the upper limit method with the presumed failure mechanism.
However, the above studies are aimed at the simple situation of the single layer overlying soil and the existing researches have not involved the situation that the anchor plate is covered by different soil layers. For this reason, this paper is primarily aimed to extend the analysis of WANG et al [38] for the ultimate pull-out force under single-layer soil to the variation analysis of the ultimate pullout force under 2-layer soil. Based on the nonlinear MC failure criterion, the kinematic admissible velocity field of the 2-layer soil above horizontal shallow strip anchor plate is constructed. According to the principle of virtual power, the power balance equation of the ultimate pullout force of the shallow strip anchor plate is obtained, and the function of failure curve is obtained by applying the variation principle. By MATLAB programming, the ultimate pullout force under specific parameters is calculated, and thus trends of ultimate pullout force and failure mechanism under different geometrical parameters are analyzed. In order to verify the effectiveness of our method, we make use of the existing cases in which the overlying layer is degraded into single-layer soil for comparison and analysis.
2 Nonlinear failure criteria
According to the Mohr-Coulomb criterion, when the geomaterials are destroyed, the normal stress is linear with the shear stress on the failure surface. However, a large number of experiments [32–37] have proved that, for geotechnical materials, Mohr failure envelope in the plane is a convex curve, as shown in Figure 1.
Figure 1 Nonlinear MC failure criterion
Obviously, there is a nonlinear relationship between the normal stress and the shear stress on the failure surface. According to Refs. [39–44], the nonlinear Mohr failure envelope, that is, the nonlinear failure criterion is expressed with stress as:
(1)
where C0 is the initial cohesion (C0 ≥ 0), σt is the uniaxial tensile strength of soil (σt ≥ 0) and both can be determined by the test. In the molar plane σn–τ, they represent the intercept of the failure envelope on the vertical axis and the horizontal axis respectively; m is the dimensionless nonlinear coefficient related to the nature of the geotechnical material, which describes the bending degree of failure envelope, and m≥1. When m=1, the curve is degraded to a straight line, and the nonlinear failure criterion is transformed to the linear Mohr-Coulomb failure criterion. The expression is as:
(2)
where c=C0 and tan φ=C0/σt.
3 Upper bound limit analysis using variation method for pullout force of horizontal shallow strip anchor plate with 2-layer soil
3.1 Analysis model of overlying 2-layer soil
For horizontal shallow strip anchor plate, it can be considered a plane-strain problem. Taking the anchor plate of unit length as the object of study, it is assumed that the soil covering the anchor plate is two layers of homogeneous ideal rigid-plastic geomaterials with different properties, which obeys the nonlinear Mohr-Coulomb failure criterion and an associated flow rule. The anchor plate is a rigid body, and there is no relative sliding between the layers mass. Thus, a corresponding curved failure mechanism in the limit state of the anchor plate is constructed, as shown in Figure 2. The failure surface equations of the soil above the anchor plate are f1(x) and f2(x); the damaged width on the surface is 2L2; the damaged width at the soil interface is 2L1; the width of the anchor plate is 2d; unit weights of two kinds of soil are γ1 and γ2, respectively. According to the limit analysis method, the kinematic admissible velocity field is established and the direction is upward. And taking the midpoint of the anchor plate as the origin, Cartesian coordinate system was built.
The curved failure surface of the soil could be considered a thin deformed layer with a certain thickness w. The shear stress and normal stress on the thin deformed layer satisfy the nonlinear Mohr-Coulomb strength criterion, and in principal stress space, the plastic potential surface is coincided with the strength yield surface. According to the associated flow rule, the following could be obtained:
(3)
According to the plastic potential theory [45]:
(4)
where εij is the plastic strain rate in the deformable structure; σij is the stress; δ is the plastic potential function and λ is the plastic factor.
Figure 2 Failure mechanism of 2-layer soil above a strip anchor plate
According to the above formula, the plastic strain rate on the thin layer is shown as follows:
(5)
From Figure 2, the plastic strain rate on the thin layer of failure surface can also be expressed as follows [46–48]:
(6)
where
As a result, the expressions of normal stress and shear stress are:
(7)
Through the superposition of the dissipation power generated by normal stress and shear stress, the dissipation power of internal energy at any point on the failure surface could be obtained [44–48]:
(8)
From the geometric relation and the physical and mechanical meaning of the failure mechanism of the anchor plate, it could be judged that the above equation satisfies D<0 in the interval [d, L2]. Taking the symmetry of the failure mechanism into account, the dissipation power of internal energy on the separated thin layer could be obtained through the integration of the above equation along the entire failure curve. Due to the different mechanical properties of the upper and lower layers, the integrations are as follows:
(9)
In the velocity field, the soil mass as a rigid body moves upward and the power of its gravity can be expressed by its volume as follows:
(10)
where γ1 is the unit weight of lower soil mass and γ2 is the unit weight of upper soil mass, kN/m3.
The power of the surface load q is:
(11)
The power of the pull force Pu on the anchor plate is:
(12)
According to the principle of virtual power, the dissipation power of internal energy is equal to that of external force, and thus a balance equation is established:
(13)
Taking Eqs. (9)–(12) into Eq. (13):
(14)
and
(15)
(16)
According to the upper bound theorem of limit analysis, the load determined by the equation of virtual power must be greater than the real load for any kinematic admissible velocity field, that is, the load value determined by the real velocity field is the closest to the real solution. From the expression of Pu, it can be seen that the extreme value of the ultimate pull force Pu is completely determined by two independent functions of Ψ1 and Ψ2. In addition, Ψ1 and Ψ2 are the simplest integral-type functions. Through the variation calculation, the problem of extreme value can be transformed to the definite problem of solving the Euler equation under the boundary condition. The Euler equations corresponding to Ψ1 and Ψ2 are shown as follows respectively:
(17)
(18)
Considering the consistency of f1(x) and f2(x), how to solve f1(x) is taken as an example. By taking the partial derivatives of Ψ1 at f1(x) and f1'(x), the following equations could be obtained:
(19)
After the integration of Eq. (17) and the substitution of Eq. (19), Eq. (20) could be as follows:
(20)
That is:
(21)
After being integrated,
(22)
Similarly,
(23)
(24)
where c1, c2, c3 and c4 are integral constants which can be determined by the boundary conditions.
As shown in Figure 2, the displacement boundary conditions are:
(25)
As the shear stress on the earth surface is equal to 0, the microelement of the surface is taken for analysis. It could be seen the horizontal component of the stress τxy=0, which satisfies:
(26)
Among them, the shear stress component τxy on the separated layer is expressed as follows:
(27)
Thus,
(28)
and
(29)
It could be obtained as follows:
(30)
According to the failure mechanism, f2′(x)>0 is permanently established, and m2>1, so f2′(x=L2)=0, that is, c3=γ2 ×L2.
At the layers interface, the soil as a rigid body does not show relative sliding. The intersection of the soil on the failure surface satisfies the deflection compatibility, so the plastic strain rate of upper and lower layers at the intersection is equal, that is:
(31)
Through simplification,
(32)
According to Eqs. (25), (30) and (32), the six nonlinear equations, it can solve c1, c2, c3, c4, L1 and L2, six unknowns in total. With Eqs. (21) to (24) substituted and integrated, the analytical formula of ultimate force Pu is shown as follows:
(33)
3.2 Specific analysis model of 2-layer overlying soil
3.2.1 Analysis model when m1=1, m2≠1
When the nonlinear coefficient m=1, the nonlinear Mohr-Coulomb strength criterion is transformed into a linear criterion, and the curved failure surface is degenerated into a flat surface. For the 2-layer soil mass, the failure mechanism has the following three cases.
When m1=1 and m2≠1, the lower soil mass obeys the linear Mohr-Coulomb failure criterion, and the failure mechanism is an oblique line. The upper soil mass obeys the nonlinear criterion and the failure mechanism is a curve.
For the lower soil mass, according to failure criterion, the slope of the tangent line is as follows:
(34)
So, the function of failure line function is:
(35)
where b1 is the undetermined coefficient and can be decided by the following boundary conditions:
(36)
Thus,
(37)
The function of failure line equation is finally as follows:
(38)
The dissipation power of internal energy of the lower soil mass is:
(39)
where l1 is the length of the failure line.
The gravity power of the lower soil mass is:
(40)
The solution to the dissipation power of internal energy and gravity power of the upper layer is similar to the above. Thus, by using the virtual power principle to establish the balance equation, the pullout force could be obtained as follows:
(41)
Known from the above,
(42)
(43)
where c3 and c4 are the undetermined coefficients and can be determined by the following boundary conditions.
(44)
The shear stress on the earth surface is equal to 0, and the failure mechanism is symmetric, so there is:
(45)
According to Eqs. (44) and (45), c3, c4 and L2 could be solved.
After substituting the function expression into Eq. (41), the ultimate pullout force is:
(46)
3.2.2 Analysis model when m2=1 and m1≠1
When m2=1 and m1≠1, the situation is contrast to the failure mechanism in Section 3.2.1. The lower soil mass obeys the nonlinear criterion and the failure mechanism is a curve. The upper soil mass obeys the linear criterion and the failure mechanism is a straight line.
The dissipation power of internal energy and gravity power in the lower soil mass are obtained in a similar way, and the dissipation power of internal energy and gravity power in the upper soil mass are:
(47)
By the principle of virtual power, a balance equation is established and the expression of pullout force Pu is obtained:
(48)
Known from the above,
(49)
(50)
where c1 and c2 are the undetermined coefficients and can be determined by the following boundary conditions.
(51)
And at the intersection,
(52)
where k2 is the slope of the failure mechanism of upper soil mass and k2 = σt2/C02.
According to Eqs. (51) and (52), c1, c2 and L1 could be solved.
The failure mechanism of the upper soil mass is:
(53)
where b2 is the undetermined coefficient and can be determined by the boundary conditions.
(54)
After substituting the function formula into Eq. (48) and the pull-out force can be obtained.
(55)
3.2.3 Analysis model when m1 =m2 =1
When m1 =m2 =1, the 2-layer soil above the horizontal strip anchor plate obeys the linear failure criterion. Thus, functions of the failure mechanism can be set as:
(56)
where k1=σt1/C01 and k2=σt2/C02; b1 and b2 are the undetermined coefficients and could be determined by boundary conditions:
(57)
The dissipation power of internal energy and gravity power in the lower soil mass are:
(58)
The dissipation power of internal energy and gravity power in the lower soil mass are obtained from the former, and the dissipation power of internal energy and gravity power in the upper soil mass are:
(59)
where l1 and l2 represent the length of failure line of the upper and lower soil mass, respectively.
Therefore, the expression of pullout force is:
(60)
3.3 Basic analysis model of single-layer overlying soil
When the parameters of the double-layer soil material are the same, the model of 2-layer soil is degraded into the model of the overlying single- layer soil. At this time, m1=m2, C01=C02 and σt1=σt2.
For the anchor plate covered with single-layer soil, the corresponding curved failure mechanism under the limit state of anchor plate is shown in Figure 3. The function of failure surface of the soil above the anchor plate is f(x); the damage width on the earth surface is 2L; the width of the anchor plate is 2d; the depth is H; the ultimate pull-out force is Pu, and the unit weight of soil is γ. According to the limit analysis method, the kinematic admissible velocity field is established and the velocity is v, whose direction is vertical upwards. Take the midpoint of the anchor plate as the origin to establish the Cartesian coordinate system.
Figure 3 Failure mechanism of single-layer soil above a shallow strip anchor plate
According to Eq. (8), the dissipation power of internal energy on the failure curve is:
(61)
The gravity power of the damaged soil is:
(62)
The power of surface overload is:
(63)
The power of ultimate pullout force is:
(64)
Therefore, according to the principle of virtual power to establish a balance equation, the power of external force is equal to that of internal energy:
(65)
Taking Eqs. (61)–(64) into Eq. (65), the ultimate pull force Pu is expressed as:
(66)
and
(67)
According to the upper bound theorem of limit analysis, the ultimate pullout force of the anchor plate determined by Eq. (67) should be greater than that of the real anchorage plate. Therefore, a minimum value should be sought in all solutions to approximate the real solution. From Eq. (66), it could be seen that the magnitude of the ultimate pullout force Pu is completely determined by the function Ψ, and Ψ is a function of f(x). So the extreme value of Pu can be obtained according to the Euler equation. Thus,
(68)
Taking the partial derivatives of Ψ at f(x) and f '(x), the following equations are obtained:
(69)
Take the integration of Eq. (69) into Eq. (68),
(70)
After the integration,
(71)
where c1 and c2 are undetermined constants and can be determined by the boundary conditions.
(72)
As the shear stress on earth surface is equal to 0, according to the force balance among the microelements of earth surface, the horizontal component of the shear stress τxy=0, that satisfies:
(73)
where the component of shear stress τxy expression on the separated thin layer is as follows:
(74)
where
(75)
Substituting Eq. (74) into Eq. (75),
(76)
According to the failure mechanism, it could be known that f ′(x)> 0 is permanent and m> 1, then f ′(x=L)=0, that is, c1=γ×L.
(77)
Through simultaneous Eqs. of (72) and (77), c1, c2 and L can be solved.
After the substitution of Eqs. (70) and (71) into Eq. (66) and the integration available, the ultimate pullout force Pu is expressed as follows:
(78)
4 Comparisons and analysis
Under the linear MC criterion, the model of overlying 2-layer soil is degraded into that of single-layer soil. Compared with the existing study of ZHAO et al [18], the results of the ultimate pullout force and failure mechanism are shown in Figure 4.
It can be seen from Figure 4 that when the nonlinear MC criterion is degraded to the linear MC criterion, the failure mechanism based on the variation method will be degraded into a simple linear failure mechanism, and the calculation result of ultimate pullout force is exactly the same as that of the simple failure mechanism of ZHAO et al [18].
Under the nonlinear MC criterion, the model of overlying 2-layer soil is degraded into a model of single-layer soil. The comparison results of ultimate pullout force and failure mechanism with the upper bound model of single-layer overlying soil of ZHAO et al [18] and the variation method of WANG et al [38] are shown in Figure 5.
Figure 4 Comparison of ultimate pullout force and failure mechanism of single-layer soil above strip anchor plate under linear MC criterion:
It can be seen from Figure 5 that when the 2-layer overlying soil is degraded into single-layer soil, the model of ultimate pull-out force in this paper is degraded into a model of single-layer. And the calculation results of the variation model in this paper are exactly the same as those of WANG et al [38]. Compared with the calculation results of the rigid limit analysis method of ZHAO et al [18], the variation method is better than the rigid upper bound method in the shallow buried range, and the range of real failure mechanism decreases significantly with the increase of nonlinearity of the failure criterion.
5 Analyses of parameters and failure mechanism
5.1 Influence of geotechnical parameters on ultimate pullout force Pu
According to Eq. (33), there are many factors affecting the ultimate pull-out force Pu, and the parameters related to the soil mass material are mainly σt, γ, C0, and m. It has been shown that the influence of the dimensionless parameter m on the ultimate pullout force is more significant [18, 38]. The following part focuses on the influence of relative value of single parameter of the soil mass on its ultimate pullout force. For other parameters, only the relative magnitudes of parameters of the upper and lower soil mass are considered. In Eq. (33), it assumes that the half-width of the anchor plate d=1.0 m, the surface overload q=0–80 kPa, the uniaxial tensile strength of lower soil mass σt1=50 kPa, the uniaxial tensile strength of upper soil mass σt2=(0.6–1.4)σt1, the unit weight of lower soil mass γ1=20 kN/m3, the unit weight of upper soil mass γ2=(0.8–1.2)γ1, the initial soil cohesion of lower soil mass C01=20 kPa, the initial soil cohesion of upper soil mass C02=(0.5–1.5)C01 and the depth H1=H2=3.0 m, respectively. When the nonlinear coefficient m=1.0–3.0, the variation curves of ultimate pullout force versus single parameters are plotted in Figures 6–14.
Figure 5 Comparison of ultimate pullout force and failure mechanism of single-layer soil above strip anchor plate under nonlinear MC criterion:
It can be seen from Figures 6–14 that the nonlinear coefficient m has a significant effect on the ultimate pullout force, and with the increase of the nonlinear coefficient m, the ultimate pullout force decreases while the influence trend is more and more gentle.
Figure 6 Variation curve of ultimate pullout force versus m1
Figure 7 Variation curve of ultimate pullout force versus ratio of γ
Figure 8 Variation curve of ultimate pullout force versus ratio of C0
Figure 9 Variation curve of ultimate pullout force versus friction angle of soil mass
Figure 10 Variation curve of ultimate pullout force versus q
It also can be seen from Figures 7–10 that under the fixed condition of the depth and the geotechnical parameters of lower soil mass, the ultimate pullout force increases when the ratios of unit weight of the soil mass γ2/γ1, the ratio of initial cohesion C02/C01, the ratio of dimensionless parameter (C02/σt2)/(C01/σt1) and the surface overload q increase.
Figure 11 shows that under the fixed condition of the depth and the geotechnical parameters of lower soil mass, with the increase of the nonlinear coefficient m, the ultimate pullout force increases with the ratio of the uniaxial tensile strength σt2/σt1. But there are different trends when the nonlinear coefficient m changes more and more largely. With the increase of the ratio of σt2/σt1, Pu monotonically under the small change of m, firstly decreases and then increases under a larger change of m, and increases monotonically under a much larger change of m.
Figures 12–14 show that under fixed conditions of the geotechnical parameters of lower soil mass and different depths, when the unit weight of geomaterials, the initial cohesion (C02 or C01) and the dimensionless parameters (C02/σt2 or C01/σt1) have a larger value, the increase of depth could enhance the ultimate pullout force of anchor plate.
Figure 11 Variation curve of ultimate pullout force versus ratio of σt2/σt1
Figure 12 Variation curve of ultimate pullout force versus depth under different conditions of unit weight of soil:
All in all, the parameter analysis above shows that the ultimate pullout force of the 2-layer soil overlying a shallow strip anchor plate is affected by geotechnical parameters of geomaterials greatly, and it is important to obtain the accurate geotechnical parameters in engineering practice for the correct evaluation of ultimate pullout capacity of the strip anchor plate.
Figure 13 Variation curve of ultimate pullout force versus depth under different conditions of uniaxial tensile strength:
Figure 14 Variation curve of ultimate pullout force versus depth under different conditions of initial cohesion:
5.2 Influence of geotechnical parameters on failure mechanism of 2-layer soil
In order to analyze the failure mechanism of the 2-layer soil above a shallow strip anchor plate in the limit state, taking into account the relative size of geotechnical parameters, i.e., the relative strength of upper and lower soil mass, the failure mechanisms with different ratios of geotechnical materials are plotted as Figures 15–23. Among the parameters, the uniaxial tensile strength of lower soil mass σt1=50 kPa, the uniaxial tensile strength of upper soil mass σt2=(0.6–1.4)σt1, the unit weight of lower soil mass γ1=20 kN/m3, the unit weight of upper soil mass γ2=(0.8–1.2)γ1, the initial cohesion of lower soil mass C01=20 kPa, the initial cohesion of the upper soil C02=(0.5–1.5)C01, the surface overload q=0–80 kPa, the nonlinear coefficient of lower soil mass m1=2.0 and the nonlinear coefficient of upper soil mass m2=1.0–3.0.
As shown in Figures 15–23, the nonlinear strength parameters, including the dimensionless coefficient m, initial cohesion C0, uniaxial tensile strength of soil mass σt, the ratio of initial cohesion and uniaxial tensile strength of soil mass C0/σt ratio, and the ratio of the relative strength parameters of upper and lower soil such as the ratio of initial cohesion C02/C01 and uniaxial tensile strength of soil mass σt2/σt1, have a significant effect on the failure mechanism of 2-layer soil above the anchor plate. The failure width L on the earth surface decreases with the increase of the nonlinear parameter m. And when m=1, the failure criterion is degraded to the linear MC criterion and the failure curve is a straight line.
Figure 15 Influence of nonlinear coefficient
Figure 16 Influence of unit weight of soil
Figure 17 Influence of initial cohesion
Figure 18 Influence of friction angle of soil mass
The failure width on earth surface decreases with the increase in the ratio of unit weight of soil mass, but the decreasing trend is gentle, as shown in Figure 16.
The failure width on earth surface increases with the increase in the ratio of initial cohesion of soil mass and the ratio of dimensionless parameters C0/σt, and the increasing trend is significant, as shown in Figures 17 and 18.
Figure 19 Influence of surface overload
Figure 20 Influence of uniaxial tensile strength
Figure 21 Influence of depth under different conditions of unit weight of soil:
Figure 22 Influence of depth under different conditions of uniaxial tensile strength of soil mass:
Figure 19 shows that the failure width does not change with the increase in surface overload. From Eqs. (21)–(24), it can be seen that the failure curves of the 2-layer soil above a shallow strip anchor plate are independent of the surface overload q. It is related to the variation model adopted in this paper. In the practical engineering, the surface overload directly affects the stress-strain conditions of the underlying geomaterials, which is related to the failure mechanism and model of the anchor plate under the limit state. Therefore, it needs further studies on the simplification of the failure mechanism and the range of the anchor plate.
In Figure 20, the failure width decreases with the increase in the uniaxial tensile strength of the soil mass, but the decreasing trend is gentle.
As shown in Figures 21–23, when the unit weight, uniaxial tensile strength and initial cohesion of upper and lower soil mass are fixed, the change of the failure width is minor with the change of the thickness of single layer under the fixed condition of total buried depth.
Figure 23 Influence of depth under different conditions of initial cohesion:
All in all, during the uplifting process, under the limit state, the failure range of the overlying 2-layer soil decreases with the increase in the nonlinear parameter m, unit weight of soil γ and uniaxial tensile strength of soil mass σt, and increases with the increase in initial cohesion of soil C0 and dimensionless parameter C0/σt. The failure range of soil mass is irrelevant to the surface overload, which is mainly caused by the simplified variation model.
6 Conclusions
1) The ultimate pullout force of the 2-layer soil above a horizontal shallow strip anchor plate is affected by the physical and mechanical parameters of geomaterials greatly. The ultimate pullout force decreases with the increase in the nonlinear coefficient m, and the influential trend is gentler and gentler. The ultimate pullout force increases with the increase in the ratio of unit weight of upper and lower layers of soil mass γ2/γ1, the ratio of initial cohesion C02/C01, the ratio of the uniaxial tensile strength (C02/σt2)/(C01/σt1) and the surface overload q. With the increase in the nonlinear coefficient m, the ultimate pullout force decreases first and then increases with the increase in the ratio of uniaxial tensile strength σt2/σt1. Under the fixed condition of geotechnical parameters of lower soil mass and different sets of ratio of thickness of upper and lower soil mass, the increase in the thickness could enhance the ultimate pullout force of the anchor plate when the unit weight, initial cohesion (C02 or C01), dimensionless parameters (C02/σt2 or C01/σt1) of soil mass are larger.
2) When the failure surface of the soil above the anchor plate represents a shape of symmetric funnel, the nonlinear strength parameters including the dimensionless coefficient m, initial cohesion C0, uniaxial tensile strength of soil mass σt, the ratio of initial cohesion and uniaxial tensile strength C0/σt, and the relative strength parameters of the upper and lower soil layers, such as the ratio of initial cohesion C02/C01 to the uniaxial tensile strength of the soil mass σt2/σt1, have a significant effect on the failure mechanism of the 2-layer soil above the anchor plate. The range of the failure mechanism decreases with the increases in the nonlinear coefficient m, the ratio of unit weight of soil γ2/γ1, the uniaxial tensile strength of soil mass σt2/σt1 while it increases with the increases in the ratio of initial cohesion C02/C01 and the friction angle of the soil. When the unit weight, uniaxial tensile strength and initial cohesion of upper and lower soil mass are fixed, the change of the failure width is small when the thickness of single layer changes under a fixed condition of the total depth.
3) When the physical and mechanical parameters of the overlying soil are the same, the model is degraded into the model of single-layer soil and the results are in good agreement with those of the previous studies, which verifies the effectiveness of our method.
4) When m=1, the failure criterion is transformed to the linear MC criterion, and the failure curve is transformed into a straight line. In the shallow-buried range, the variation method outperforms the upper limit method.
References
[1] MEYERHOF G G, ADAMS J I. Ultimate uplift capacity of foundation [J]. J Can Geotech, 1968, 5(4): 225–244.
[2] DAS B M. Model tests for uplift capacity of foundations in clay [J]. Soils and Foundations, 1978, 18(2): 17–24.
[3] MURRAY E J, GEDDES J D. Uplift of anchor plates in sand [J]. Journal of Geotechnical Engineering, ASCE, 1987, 113(3): 202–215.
[4] QIAN Ping-yi, LIU Zu-de. Distortion and failure character of shallow buried inclined anchors [J]. Chinese Journal of Geotechnical Engineering, 1992, 14(1): 62–66. (in Chinese)
[5] HE Si-ming. Study on bearing capacity of uplift anchor foundation [J]. Underground Space, 2002, 22(2): 145–148. (in Chinese)
[6] ILAMPARUTHI K, DICKIN E A, MUTHUKRISHNAIAH K. Experimental investigation of the uplift capacity of circular plate anchors in sand [J]. J Can Geotech, 2002, 39: 648–664.
[7] CHU Xiao-feng, LI Zhi-gang, WANG Ren, ZHU Chang-qi. The test research of anchor‘s uplift behavior in calcareous sand [J]. Geomaterial Mechanics, 2002, 23(3): 368–664. (in Chinese)
[8] ZHU Chang-qi, CHU Xiao-feng. Calcareous sand in the limits of plate anchor uplift force calculation [J]. Rock and Geomaterial Mechanics, 2003, 24: 153–158. (in Chinese)
[9] DING Pei-min, XIAO Zhi-bin, ZHANG Qi-lin, QIU Tao. Uplift capacity of plate anchors in sand [J]. Journal of Building Structures. 2003, 24(5): 82–91. (in Chinese)
[10] DICKIN E A, LAMAN M. Uplift response of strip anchors in cohesionless soil [J]. Advances in Engineering Software, 2007, 38(9): 618–625.
[11] LIU Wen-bai. The bearing behavior and calculation of the anti-uplift foundation [M]. Shanghai: Shanghai Jiao Tong University Press, 2007. (in Chinese)
[12] ZHANG Ning, WU Huai-na, SHEN Jack Shui-Long, HINO Takenori, YIN Zhen-yu. Evaluation of the uplift behavior of plate anchor in structured marine clay [J]. Marine Georesources & Geotechnology, 2017, 35(6): 758–768.
[13] GIAMPA JOSEPH R, BRADSHAW AARON S, SCHNEIDER JAMES A. Influence of dilation angle on drained shallow circular anchor uplift capacity [J]. International Journal of Geomechanics, 2017, 17(2): 04016056.
[14] LIU Hua-qiang, HUANG Jing-zhong. Vertical uplift capacity of horizontal plate anchors [J]. Geotechnical Engineering Technique, 2007, 21(1): 25–27. (in Chinese)
[15] GHALY A, HANNA A. Ultimate pullout resistance of single vertical anchors [J]. J Can Geotech, 1994, 31: 661–672.
[16] MERIFIELD R S, LYAMIN A V, SLOAN S W. Three-dimensional lower bound solutions for the stability of plate anchors in sand [J]. Géotechnique, 2006, 56(2): 123–132.
[17] KOUZER K M, KUMAR J. Vertical uplift capacity of equally spaced horizontal strip anchors in sand [J]. International Journal of Geomechanics, ASCE, 2009, 9(5): 230–236.
[18] ZHAO Lian-heng, LI Liang, YANG Feng, LIU Xiang. Joined influences of nonlinearity and dilation on the ultimate pullout capacity of horizontal shallow plate anchors by energy dissipation method [J]. International Journal of Geomechanics, ASCE, 2011, 11(3): 195–201.
[19] KOUZER K M, KUMAR J. Vertical uplift capacity of two interfering horizontal anchors in sand using an upper bound limit analysis [J]. Computers and Geotechnics, 2009, 36(6): 1084–1089.
[20] KHATRI V N, KUMAR J. Vertical uplift resistance of circular plate anchors in clays under undrained condition [J]. Computers and Geotechnics, 2009, 36(8): 1352–1359.
[21] PARAMITA B, JYANT K. Seismic pullout capacity of inclined anchor plates in sand [J]. Geotechnical and Geological Engineering, 2017, 35(2): 679–692.
[22] PARAMITA B, ANAMITRA R. Variation of horizontal pullout capacity with width of vertical anchor plate [J]. Int J Geomech, 2016, 16(5): 06016002.
[23] PARAMITA B, JYANT K. Uplift capacity of anchors in layered sand using finite-element limit analysis: Formulation and results [J]. Int J Geomech, 2016, 16(3): 04015078.
[24] ZHAO Lian-heng, YANG Xin-ping, HUANGFu, TANG Yi-gao, HUShi-hong. Variational analysis of the ultimate pullout capacity of shallow circular anchor plates in rock foundations based on the Hoek-Brown failure criterion [J]. International Journal of Rock Mechanics and Mining Sciences, 2018,106:190–197.
[25] ZHAOLian-heng, TANG Yi-gao, HU Shi-hong, DENG Dong-ping, YANG Xin-ping. Upper bound analysis of the ultimate pullout capacity of shallow 3-D circular plate anchors based on the nonlinear mohr-coulomb failure criterion [J]. Journal of Central South University, 2018, 25(9): 25: 2272-2288.
[26] ROWE R K, DAVIS E H. The behaviour of anchor plates in sand [J]. Geotechnique, 1982, 32(1): 9–23.
[27] LIU Wen-bai, ZHOU Jian. Partical flow code numerical simulation of extended foundation under the action of uplift loading [J]. Journal of Hydraulic Engineering, 2004, 35(12): 69–76. (in Chinese)
[28] WANG Dong, HU Yu-xia, RANDOLPH M F. Three- dimensional large deformation finite-element analysis of plate anchors in uniform clay [J]. Journal of Geotechnical and Geoenvironmental Engineering, ASCE, 2010, 136(2): 355–365.
[29] YU Long, LIU Jun, KONG Xian-jing, HU Yu-xia. Three- dimensional numerical analysis of the keying of vertically installed plate anchors in clay [J]. Computers and Geotechnics, 2009, 36(4): 558–567.
[30] SU Fang-mei, LIU Hai-xiao, LI Zhou. Analysis of ultimate bearing capacity of plate anchors in clay using a coupled Eulerian-Lagrangian method [J]. Rock and Soil Mechanics, 2016, 37(9): 2728–2736. (in Chinese)
[31] EMIRLER B, BILDIK S, LAMAN M. Numerical investigation of anchor plates in layered soil [J]. International Journal of Material Science & Engineering, 2015, 2(1): 10–15.
[32] HOEK E. Strength of joined rock masses [J]. Geotcehnique, 1983, 33(3): 187–223.
[33] AGAR J G, MORGENSTERN N R, SCOTT J. Shear strength and stress-strain behavior of Athabasca oil sand at elevated temperatures and pressures [J]. Canadian Geotechnical Journal, 1987, 24(1): 1–10.
[34] CHEN W F, LIU X L. Limit analysis in soil mechanics [M]. Amsterdam: Elsevier Science, 1990.
[35] MAKSIMOVIC M. Nonlinear failure envelope for soils [J]. Jour of Geotech Eng, ASCE, 1989, 115(4): 581–586.
[36] BAKER R. Nonlinear Mohr envelopes based on triaxial data [J]. Journal of Geotechnical and Geoenvironmental Engineering, ASCE, 2004, 130(5): 498–506.
[37] HOEK E, BRAY J W. Rock slope engineering [M]. London: The Institution of Mining and Metallurgy, 1981.
[38] WANG Hong-tao, LI Shu-cai, WANG Qi, MIAO Su-jun, JIANG Bei. Limit analysis of ultimate pullout capacity of shallow horizontal strip anchor plate based on nonlinear failure criterion [J]. Engineering Mechanics, 2014, 31(2): 131–138. (in Chinese)
[39] ZHANG X J, CHEN W F. Stability analysis of slopes with general nonlinear failure criterion [J]. International Journal for Numerical and Analytical Methods in Geomechanics, 1987, 11(1): 33–50.
[40] DRESCHER A, CHRISTOPOULOS C. Limit analysis slope stability with nonlinear yield condition [J]. International Journal for Numerical and Analytical Methods in Geomechanics, 1988, 12(3): 341–345.
[41] YANG Xiao-li, YIN Jian-hua. Slope stability analysis with nonlinear failure criterion [J]. Journal of Engineering Mechanics, ASCE, 2004, 130(3): 267–273.
[42] ZHAO Lian-heng, YANG Feng, ZHANG Ying-bin, DAN Han-cheng, LIU Wei-zheng. Effects of shear strength reduction strategies on safety factor of homogeneous slope based on a general nonlinear failure criterion [J]. Computers and Geotechnics, 2015, 63: 215–228.
[43] ZHAO Lian-heng, CHENG Xiao, DAN Han-cheng, TAN Zu-ping, ZHANG Ying-bin. Effect of vertical earthquake component on the permanent seismic displacement of soil slopes based on the nonlinear Mohr-Coulomb failure criterion [J]. Soil and Foundations, 2017, 57(2): 237–251.
[44] TANG Gao-peng, ZHAO Lian-heng, LI Liang, CHEN Jing-yu. Combined influence of nonlinearity and dilation on slope stability evaluated by upper-bound limit analysis [J]. Journal of Central South University, 2017, 24(7): 1602– 1611.
[45] ZHANG Xue-yan. Geotechnical plastic mechanics [M]. Beijing: China Communication Press, 1993. (in Chinese)
[46] FRALDI M, GUARRACINO F. Limit analysis of collapse mechanisms in cavities and tunnels according to the Hoek-Brown failure criterion [J]. International Journal of Rock Mechanics & Mining Sciences, 2009, 46(4): 665–673.
[47] FRALDI M, GUARRACINO F. Analytical solutions for collapse mechanisms in tunnels with arbitrary cross sections [J]. International Journal of Solids and Structures, 2010, 47(2): 216–223.
[48] FRALDI M, GUARRACINO F. Limit analysis of progressive tunnel failure of tunnels in Hoek–Brown rock masses [J]. International Journal of Rock Mechanics & Mining Sciences, 2012, 50(2): 170–173.
(Edited by YANG Hua)
中文导读
基于非线性MC破坏准则的上覆双层土体水平浅埋条形锚板极限抗拔力变分分析
摘要:基于非线性Mohr-Coulomb强度准则及相关联的流动法则,构造出水平浅埋条形锚板上覆双层土体破坏的机动许可场;利用极限分析上限法,结合变分原理,推导出了水平浅埋条形锚板上覆双层土体的极限抗拔力表达式及其对应破裂机制;当上覆土层退化为单层土体时,极限抗拔力计算模型可以退化为既有单层土体计算模型;通过单层土体变分法计算结果与刚体极限分析法计算结果的对比,进一步证明了本文方法的正确性;在此基础上,分析了岩土物理力学参数变化对上覆双层土体锚板极限抗拔力与土体破裂机制的影响。计算表明:水平浅埋条形锚板上覆双层土体极限抗拔力和破坏模式受岩土体非线性物理力学特性参数的影响非常显著,工程实际中准确获取双层土体的岩土体物理力学特性参数对于正确评估锚板极限抗拔特性非常重要。
关键词:浅埋条形锚板;双层土体;极限抗拔力;变分分析;非线性破坏准则
Foundation item: Project(51478477) supported by the National Natural Science Foundation of China; Project(2016CX012) supported by the Innovation-Driven Project of Central South University, China; Project(2014122006) supported by the Guizhou Provincial Department of Transportation Foundation, China
Received date: 2017-06-29; Accepted date: 2018-01-29
Corresponding author: NIE Zhi-hong, PhD, Associate Professor; Tel: +86-13973172613; E-mail: 707934640@qq.com; ORCID: 0000- 0002-9660-1737; ZHAO Lian-heng, PhD, Professor; Tel: +86-13755139425; E-mail: zlh8076@163.com; ORCID: 0000-0002-8406-5973
Abstract: Based on the nonlinear Mohr-Coulomb failure criterion and an associated flow rule, a kinematic admissible velocity field of failure mechanism of the 2-layer soil above a shallow horizontal strip anchor plate is constructed. The ultimate pull-out force and its corresponding failure mechanism through the upper bound limit analysis according to a variation principle are deduced. When the 2-layer overlying soil is degraded into single-layer soil, the model of ultimate pullout force could also be degraded into the model of single-layer soil. And the comparison between results of single-layer soil variation method and those calculated by rigid limit analysis method proves the correctness of our method. Based on that, the influence of changes of geotechnical parameters on ultimate pullout forces and failure mechanism of a shallow horizontal strip anchor with the 2-layer soil above are analyzed. The results show that the ultimate pull-out force and failure mechanism of a shallow horizontal strip anchor with the 2-layer soil above are affected by the nonlinear geotechnical parameters greatly. Thus, it is very important to obtain the accurate geotechnical parameters of 2-layer soil for the evaluation of the ultimate pullout capacity of the anchor plate.
- Variation analysis of ultimate pullout capacity of shallow horizontal strip anchor plate with 2-layer overlying soil based on nonlinear M-C failure criterion
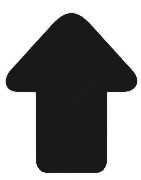