- Abstract:
- 1 Introduction▲
- 2 Materials and meth...▲
- 3 Results and discus...▲
- 4 Conclusions▲
- References
- Figure
- Figure 1 XRD patterns (a), Raman (b) and FTIR spectra (c) of MnO2 and CoMn2O4 products, high-resolution XPS spectra of Mn 2p (d), Co 2p (e) and O 1s (f) core levels in CoMn2O4 product obtained after 1 h reaction (denoted as CoMnO-1 h)
- Figure 2 SEM images of MnO2 precursor (a, b), CoMnO-10 min (c), CoMnO-1 h (d) products
- Figure 3 TEM (a, c) and HRTEM (b, d) images:
- Figure 4 N2 adsorption/desorption isotherms (a) and pore size distribution (b) of MnO2 precursor and CoMn2O4 samples prepared after 10 min (CoMnO-10 min) and 1 h (CoMnO-1 h)
- Figure 5 Electrochemical property of hierarchical spherical MnO2 material:
- Figure 6 Electrochemical property of hierarchical spherical CoMn2O4 material:
ARTICLE
J. Cent. South Univ. (2019) 26: 1481-1492
DOI: https://doi.org/10.1007/s11771-019-4104-9
Facile synthesis of hierarchically structured manganese oxides as anode for lithium-ion batteries
DENG Zhao(邓兆)1, HUANG Xing(黄兴)1, ZHAO Xu(赵煦)2,CHENG Hua(程化)3, WANG Hong-en(王洪恩)1
1. State Key Laboratory of Advanced Technology for Materials Synthesis and Processing,Wuhan University of Technology, Wuhan 430070, China;
2. Institute of Chemical Materials, China Academy of Engineering Physics, Mianyang 621900, China;
3. Department of Materials Science and Technology, Southern University of Science and Technology, Shenzhen 518055, China
Central South University Press and Springer-Verlag GmbH Germany, part of Springer Nature 2019
Abstract:
Developing high-performance lithium ion batteries (LIBs) using manganese oxides as anodes is attractive due to their high theoretical capacity and abundant resources. Herein, we report a facile synthesis of hierarchical spherical MnO2 containing coherent amorphous/crystalline domained by a simple yet effective redox precipitation reaction at room temperature. Further, flower-like CoMn2O4 constructed by single-crystalline spinel nanosheets has been fabricated using MnO2 as precursor. This mild methodology avoids undesired particle aggregation and loss of active surface area in conventional hydrothermal or solid-state processes. Moreover, both MnO2 and CoMn2O4 nanosheets manifest superior lithium-ion storage properties, rendering them promising applications in LIBs and other energy-related fields.
Key words:
manganese oxides; nanostructures; anode materials; lithium ion batteries; electrochemistry;
Cite this article as:
DENG Zhao, HUANG Xing, ZHAO Xu, CHENG Hua, WANG Hong-en. Facile synthesis of hierarchically structured manganese oxides as anode for lithium-ion batteries [J]. Journal of Central South University, 2019, 26(6): 1481-1492.
DOI:https://dx.doi.org/https://doi.org/10.1007/s11771-019-4104-91 Introduction
Rechargeable lithium-ion batteries (LIBs) have been widely used in portable electronic devices because of their commercialization [1]. Now their performances are being further improved for emerging applications in (hybrid) electric vehicles (EVs), smart grids and storage of electricity from various renewable sources [2]. This poses strict requirements on searching new high-performance electrode materials with high capacity, excellent rate capability, good safety, low cost and environmental cleanness.
Graphite has been employed as anode for commercial LIBs for more than two decades. However, its relatively low theoretical capacity (372 mA·h/g), poor rate property and inferior safety have restricted its future applications in EVs. Several kinds of alternative anode materials are currently being investigated, such as silicon (Si) [3], tin (Sn)/antimony (Sb) [4] and their oxides/sulfides [5, 6], and transition metal oxides/sulfides/ selenides [7, 8].Particularly, manganese oxides (e.g. MnO2 [9], Mn2O3 [10], Mn3O4 [11] and MnO [12]) have received increasing research attention due to their high capacities, diverse atomic configurations and multiple valence states of Mn, as well as natural abundance and environmental friendliness [13]. The rich composition and phase structures of manganese oxides, as well as abundance of Mn in earth crust have rendered them promise alternative anodes in LIBs. Among them, MnO2 has been explored as one of the most promising anode materials for LIBs due to its high capacity, good safety and low cost. Besides MnO2, some complex oxides from combination of two transition metal oxides, or a transition metal oxide and a post-transition metal oxide, such as CoMn2O4, has recently attracted significant interest as an anode for LIBs as well because of their complementarities and synergy in the charge/discharge process for the two transition metal oxides [14].
However, the low ionic/electronic conductivity of MnO2 and CoMn2O4 has limited their Li-ion storage property especially at high charge/discharge rates. Moreover, the first discharge (lithiation) process of MnO2 and CoMn2O4 usually involves the cleavage of Mn-O/Co-O bonds and amorphization of the host crystal structure, giving rise to poor reaction kinetics and large electrode polarization. Thus, the rate property and cycling stability of MnO2 and CoMn2O4 need to be further improved through rational structure/composition regulation. Various low-dimensional MnO2 and CoMn2O4 nanostructures, such as nanoparticles [15], nanowires [16], nanotubes [17], and nanorods [18] have been delicately designed and synthesized with improved electrochemical properties. Further, conductive carbon (e.g. graphene [19] or carbon nanotubes [20]) has also been blended with MnO2 or CoMn2O4 to further enhance their electrical conduction. Most recently, phase hybridization engineering has been proposed to be another effective strategy to enhance the electrochemical performance of oxide electrode materials [7, 8, 21].
However, low-dimensional nanostructures suffer from easy aggregation and loss of active electrochemical surface due to their high surface energy. In comparison, hierarchically (porous) nanoarchitectures exhibit some unique structure advantages of high surface area and packing density with less self-aggregation [22]. In addition, conventional synthesis approaches for MnO2 or CoMn2O4 nanostructures usually involve hydrothermal and/or solid-state reaction processes [23, 24], which are energy costive and occasionally lead to undesired grain growth with reduced active surface. Thus, exploration of more effective synthetic strategy for hierarchical MnO2/CoMn2O4 nanostructures remains significant and challenging.
In this work, we report the facile synthesis of hierarchical spherical MnO2 composed of nanosheets via a one-pot wet-chemical route at room temperature. Interestingly, the resultant MnO2 nanosheets contain abundant crystalline/amorphous domains, leading to formation of many nanoscale coherent heterojunctions. Further, hierarchical flowerlike CoMn2O4 constructed by ultrathin single-crystal nanosheets are also prepared on a large scale from a two-step redox reaction at room temperature. Electrochemical measurements indicate that both MnO2 and CoMn2O4 samples manifest superior lithium-ion storage property. The present methodology may provide new routes for the synthesis of other functional compounds with phase hybridization and morphology engineering.
2 Materials and methods
2.1 Materials synthesis
2.1.1 Synthesis of hierarchical spherical MnO2
MnSO4·H2O (1.014 g) and KMnO4 (0.632 g) were respectively dissolved into 40 mL deionized (DI) water under magnetically stirring. Then, the two solutions were mixed under strongly stirring at room temperature, and then kept static for another 15 min. During mixing, abundant brown black precipitates were produced, suggesting the strong reaction tendency between KMnO4 and MnSO4. This observation is in accordance with the estimation of the large standard Gibbs free energy change (ΔG0) of –269.3 kJ/mol calculated based on the redox potential difference between MnO4–/MnO2 and MnO2/Mn2+ redox pairs. After reaction, the resultant precipitates were thoroughly rinsed with DI water to remove excess ions and then harvested by filtering, and finally dried at 60 °C for 12 h in air.
2.1.2 Synthesis of hierarchical flowerlike CoMn2O4
The amorphous MnO2 precursor (0.174 g) was dispersed into 10 mL DI water, followed by dissolution of CoCl2·6H2O (0.238 g) along with stirring for 15 min. NaBH4 (0.696 g) was dissolved in another 10 mL DI water, followed by addition of NaOH to adjust the pH of the NaBH4 solution to 11–12. Then, the NaBH4 solution was dropwise added into the MnO2 and CoCl2·6H2O mixture under vigorous stirring. After addition, the mixture was allowed for further reaction for different durations (10 min–1 h). Note that some Mn-O bonds could be cleaved, and new Co-O bonds were formed during reaction between amorphous MnO2 and Co2+ under reduction conditions. Then, the resultant CoMn2O4 products were thoroughly washed with DI water, followed by collection via filtration, and finally dried at 60 °C for 12 h in vacuum. For simplicity, the CoMn2O4 products prepared after solution reaction for 10 min and 1 h are named as CoMnO-10 min and CoMnO-1 h, respectively.
2.2 Materials characterization
X-ray diffraction (XRD) patterns were recorded on a D8 advance X-ray diffractometer using Cu Kα radiation (λ=1.54056 ). The morphology of the samples was observed by a Hitachi S-4800 field-emission scanning electron microscope (SEM). Transmission electron microscopy (TEM) images, selected-area electron diffraction (SAED) patterns, high-resolution TEM (HRTEM) micrographs, and fast Fourier transform (FFT) patterns were acquired using a JEOL 2100F electron microscope at 200 kV. The surface element electronic states were analyzed by X-ray photoelectron spectroscope (XPS, Thermo Fisher, Alpha). The binding energies (BE) for the samples were calibrated using C 1s peak from a carbon tape at 284.8 eV. N2 adsorption/desorption experiments were performed at 77 K on a Tri Star II 3020 surface area and porosity analyzer. The specific surface area of the samples was calculated using a multipoint Brunner-Emmett-Teller (BET) method. Prior sorption, the samples were degassed at 100 °C for 48 h under vacuum. Fourier transform infrared spectra (FTIR) and Raman spectra were recorded on a Nexus FTIR spectrometer.
2.3 Electrochemical tests
The electrochemical tests were performed by using CR2025 coin-type cells with lithium foils as the counter/reference electrode and microporous membrane (Celgard 2400) as the separator. The working electrode was prepared by thoroughly blending a slurry containing the active material (MnO2 or CoMn2O4), acetylene black, and polyvinylidene fluoride (PVDF) binder with a mass ratio of 7:2:1 in N-methyl-2-pyrrolidone (NMP) solvent. The resultant slurry was uniformly coated on a Cu foil and dried at 120 °C for 12 h in vacuum and then cut into circular disks with an active material loading of 1.0 mg/cm2. The electrolyte was 1.0 mol/L LiPF6 dissolved in ethylene carbonate (EC) and dimethyl carbonate (DMC) with a volume ratio of 1:1. The cells were assembled in an ultrapure argon-filled glovebox with both moisture and oxygen content below 0.3×10–6. Galvanostatic discharge/charge cycling was conducted using a LAND-CT2001A within a potential window of 0.05–3.0 V vs Li+/Li at different current densities. Cyclic voltammetry (CV) curves were measured at 0.2 mV/s in a potential window of 0.05–3.0 V by an electrochemical workstation (CHI 604e, Shanghai Chenhua). Electrochemical impedance spectroscopy (EIS) was recorded on an electrochemical workstation (Autolab PGSTAT 302N) at a frequency region of 0.01–100 kHz. All the electrochemical measurements were carried out at room temperature.
3 Results and discussion
3.1 Structure and morphology characterizations of as-prepared MnO2 and CoMn2O4
XRD patterns of the as-prepared MnO2 and CoMn2O4 samples are depicted in Figure 1(a). For the MnO2 sample (bottom, black), no obvious diffraction peaks are observed, suggesting its dominantly amorphous feature. Similarly, the CoMn2O4 product prepared after 10-min reaction (denoted as CoMnO-10 min) reassembles amorphous structure (middle, red). In contrast, the CoMn2O4 product after 1 h reaction (CoMnO-1 h) is characterized by a tetragonal symmetry (space group I41/amd, JCPDS No. 77-0471) [25]. The structure of MnO2 and CoMn2O4 was further characterized by Raman and FTIR spectra. In the Raman spectra (Figure 1(b)), one noticeable peak at about 612 cm–1 is noted for the MnO2 sample, which corresponds to the symmetric stretching vibration of Mn-O bond of [MnO6] groups [26]. A similar Raman spectrum is noticed for CoMnO-10 min sample due to its amorphous structure.
Figure 1 XRD patterns (a), Raman (b) and FTIR spectra (c) of MnO2 and CoMn2O4 products, high-resolution XPS spectra of Mn 2p (d), Co 2p (e) and O 1s (f) core levels in CoMn2O4 product obtained after 1 h reaction (denoted as CoMnO-1 h)
For the CoMnO-1 h, several Raman peaks (288, 316 and 373 cm–1) are observed and can be assigned to tetragonal CoMn2O4 [27]. From the FTIR spectra (Figure 1(c)), the absorption peaks at about 3431 and 1636 cm–1 can be assigned to the vibration of O—H groups from surface adsorbed water on the MnO2/CoMn2O4 samples [28]. In addition, the absorption peaks at about 753 and 490 cm–1 can be indexed to the vibrations of Mn—O bonds [28] while the peak at about 610 cm-1 signals the Co—O vibration in CoMn2O4 [29].
The surface composition and chemical states of CoMnO-1 h were investigated using XPS. The deconvolution of Mn 2p spectrum (Figure 1(d)) indicates the presence of Mn2+ with binding energy (BE) of 2p3/2 at 641.5 eV and Mn3+ with BE of 2p3/2 at 643.2 eV [30]. High-resolution Co 2p spectrum (Figure 1(e)) can be deconvoluted into three species with BE at about 781.1, 783 and 787.2 eV for 2p3/2 orbital of Co3+ and Co2+ and satellite peak of Co2+ ions, respectively [31]. In Figure 1(f), the fitting of O 1s spectrum unveils the presence of O—Co/O—Mn bonds (about 530.4 eV) and O—H bonds (about 532.2 eV) of surface adsorbed water [32].
The morphologies of the as-synthesized MnO2 and CoMn2O4 samples are observed by SEM. Figures 2(a) and (b) reveal that the MnO2 product mainly consists of hairy spheres with diameters of about 500 nm. Each spherical particle is comprised of many nanosheets, presenting a hierarchically porous architecture. The formation of such hierarchical microspheres can be deduced as follows. Initially, abundant MnO2 nuclei were rapidly formed between the redox reaction of KMnO4 and MnSO4 upon mixing. These MnO2 nuclei aggregated into some large secondary particles due to their high surface energies. Then, the inner MnO2 nuclei in each aggregate evolved into lamellar nanostructures gradually via linkage of the edge- and corner-shared [MnO6] octahedra. Finally, hierarchically structured spheres constructed by lamellar nanosheets were obtained. From Figures 2(c) and (d), the resulting CoMn2O4 samples show flowerlike structure consisting of thin nanosheets, which roughly inherits the morphology of the MnO2 precursor. Note that the structure transition from MnO2 to CoMn2O4 may be energetically favored due to the relatively easy cleavage of some local Mn-O bonds in the amorphous MnO2. Instead, breaking Mn-O bonds in crystallized MnO2 may need to overcome a larger (Madelung) lattice energy, whereas a high- temperature solid-state reaction is often required [33].
Figure 3(a) shows that the MnO2 precursor consists of many thin nanosheets, presenting semitransparent characteristics due to their loose, porous and hierarchical structure. This feature is beneficial for conversion into CoMn2O4 by enhanced interfacial reaction with Co2+. HRTEM micrograph (Figure 3(b)) and FFT pattern (top right) taken from central area 1 (blue square) indicate the amorphous structure of the MnO2. In addition, nano-crystallites with tetragonal alpha- phase (space group I4/m (87), JCPDS No. 44-0141) are occasionally detected in bottom region 2 (yellow square) by HRTEM and FFT pattern (inset, bottom right). To the best of our knowledge, the coexistence of crystalline and amorphous domained in MnO2 has seldom been reported in literature. LI et al [34] reported the fabrication of core-shell structured a-MnO2 catalyzed by Ag+ in solution at room temperature. In their process, an Ostwald ripening mechanism was proposed for the formation of core-shell nanostructure, whereas a rigid crystalline outer shell was firstly formed, followed by crystallization of the interior core. The underlying mechanism for forming such coexisted amorphous/crystalline domained in the MnO2 nanosheets remains unclear and needs more in-depth investigation. TEM image (Figure 3(c)) validates the ultrathin nanosheet morphology of the CoMn2O4 product. The SAED pattern (inset,Figure 3(c)) suggests its tetragonal spinel structure, which agrees with the XRD result. The lattice fringes with interplanar distance of about 0.31 nm shown in HRTEM micrograph (Figure 3(d)) can be indexed to the (202) plane of tetragonal CoMn2O4. FFT pattern (inset, Figure 3(d)) depicts a clear 2D dotted pattern showing (202), (211) and (004) planes, indicating the single-crystal nature of the CoMn2O4 nanosheets.
Figure 2 SEM images of MnO2 precursor (a, b), CoMnO-10 min (c), CoMnO-1 h (d) products
Figure 3 TEM (a, c) and HRTEM (b, d) images:
Figure 4(a) presents N2 sorption isotherms of the obtained MnO2 and CoMn2O4 samples. All the isotherms show a typical type III curve with H1-type hysteresis, representing mesoporous structure containing slit-like pores formed by packing of nanosheets. The Brunner-Emmett-Teller (BET) specific surface areas of the MnO2, CoMnO-10 min and CoMnO-1 h are 339, 190 and 228 m2/g, respectively. The Barrett-Joyner-Halenda (BJH) pore size distribution curves (Figure 4(b)) unveil the existence of mesopores with size distribution centering around 3–4 nm. The high surface area, ultrathin nanosheet morphology and hierarchical organization of the MnO2 and CoMn2O4 products may render them promising application in electrochemical energy storage and conversion. As a proof-of-concept, the Li-ion storage performances of the MnO2 and CoMn2O4-1 h materials have been evaluated using CR2025 Li-half cells. Figure 5(a) depicts the cyclic voltammograms (CV) of the hierarchical MnO2 between 0.05 and 3 V at a scan rate of 0.2 mV/s. During the first cathodic process, a broad reduction peak centered around 0.7 V can be assigned to the insertion of Li+ into MnO2 framework to form LixMnO2 (x≤2) and formation of solid-electrolyte interphase (SEI) layer accompanied by electrolyte decomposition. The second strong reduction peak at about 0.2 V is due to the reduction of Mn4+ to Mn0 [35]. The peak at 1.2 V in the corresponding anodic process is associated with the re-oxidation of Mn0 to Mn2+. A couple of redox peaks are observed in the following cycles, corresponding to the continuous reduction/oxidation of Mn2+/Mn0. In addition, the CV curves in the 2nd and 3rd cycles almost overlap, implying the good electrochemical reversibility of the reaction. Figure 5(b) shows the galvanostatic discharge/charge curves of the MnO2 anode at a current density of 50 mA/g in the potential range from 0.05 to 3 V. For the first discharge, a sloping plateau between 1.2–0.6 V reflects the Li+ intercalation into MnO2 structure and SEI formation caused by the decomposition of electrolyte solvent. Another long plateau at about 0.5 V corresponds to the further lithiation of LixMnO2 by the conversion reaction with the formation of metallic Mn and Li2O species [36]. In the subsequent charge, a sloping plateau between 1–1.3 V is noted, corresponding to the decomposition of Li2O and re-oxidation of Mn into Mn2+. In the following discharge/charge cycles, both a sloping discharge and charge plateau is observed, indicating the reversible reduction and oxidation of Mn2+ and Mn. The galvanostatic discharge/charge agrees well with the CV result. The MnO2 electrode displays an initial discharge capacity of 1460 mA·h/g in the 1st cycle, which is near to its theoretical capacity (~1233 mA·h/g). The charge capacity in the 1st cycle yields 622 mA·h/g, giving an initial Coulombic efficiency (CE) of 43%. The relatively low initial CE value can be mainly ascribed to the formation of SEI with electrolyte decomposition and the change of end product into MnO (instead of MnO2) by the reaction between Li2O and Mn0 during recharge, as already confirmed in previous literature. The discharge and charge capacities of the MnO2 electrode decrease to 662 and 547 mA·h/g, with a much improved CE of 83% in the 2nd cycle. Next, the electrode exhibits slow capacity decay from the 3rd to 50th cycle, suggesting the improved electrode reversibility and stability. Figure 5(c) presents the discharge/charge curves of the MnO2 electrode at different current densities. Clearly, all the voltage profiles show sloping curves. In addition, the MnO2 anode displays reduced discharge/charge capacities with increasing current densities due to the increased polarization. Figure 5(d) shows the rate performance of the MnO2 material evaluated at different current densities. Evidently, the anode presents superior rate capability for Li+ storage with averaged capacities of 560, 359, 240 and 168 mA·h/g at current densities of 100 mA/g, 200 mA/g, 500 mA/g and 1 A/g, respectively. After switching back to 100 mA/g, a reversible capacity of 400 mA·h/g can be recovered. The long-term cycling test of the MnO2 electrode was measured at 100 mA/g for 100 cycles as shown in Figure 5(e). The materials exhibit a high initial capacity of 620 mA·h/g (2nd cycle) and slow capacity decay. The Li+ insertion/extraction is highly reversible with CE approaching unity with cycling. The capacity over 50th cycles retains 386 mA·h/g. Interestingly, a gradual capacity increase has been observed after 50 cycles, and the capacity after 100 cycles recovers to 565 mA·h/g. Similar self- improving behavior on Li+ storage capacity has also been obviously. For example, CHEN et al [35] reported that PEDOT-coated hollow MnO2 nanoboxes exhibited an increased Li+ storage capacity along with cycling, which was obviously different from the pristine MnO2 nanoboxes. They deduced that primary MnO2 particles would be separated into smaller particles, which increased the contact area between the active materials and the electrolyte and yielded an increased capacity. We assumed that a similar process might occur for our MnO2 material as well. In addition, the increasing of capacity during cycling can be attributed to 1) formation of partially decomposable polymer-like SEI film and 2) partial oxidation of Mn2+ to higher valence-state manganese oxides [9]. Moreover, the hierarchical architecture formed by thin nanosheets could well buffer the repeated volume changes during cycling. This could avoid the undesired capacity decay caused by loss of electrical contact between active material and current collector. Electrochemical impedance spectra (EIS) were further recorded to probe the electrode kinetics information. As shown in Figure 5(f), the two Nyquist plots for fresh and cycled Li-cells both contain a semicircle at high- to-medium frequency region and a sloped line at low frequency. The semicircle provides information on the Li+ transfer at electrode/electrolyte interface, while the sloping line represents the Li+ diffusion in the MnO2 phase. Interestingly, the cycled electrode has a smaller semicircle, suggesting its lower charge transfer resistance (Rct) compared to the fresh electrode, which supports the self-improving phenomenon on capacity during cycling shown in Figure 5(e). The electrochemical Li+ storage capability of the hierarchical MnO2 materials also surpasses several MnO2 nanostructures in the literature, such as coaxial CNTs/MnO2 nanotubes (500 mA·h/g after 15 cycles at 50 mA/g) [37], MnO2 nanoparticles (154 mA·h/g after 50 cycles at 100 mA/g) [38] and MnO2 nanorods (131 mA·h/g after 60 cycles at 100 mA/g) [39]. The electrochemical property of CoMn2O4 was also measured as shown in Figure 6. Cycling test at 100 mA/g reveals that the material exhibits a high initial capacity of 890 mA·h/g (2nd cycle) and slow capacity decay (Figure 6(a)). The Li+ insertion/ extraction is highly reversible with CE approaching unity with cycling. The capacity over 100 cycles can remain a high value of 260 mA·h/g. Figure 6(b) shows the rate performance of the CoMn2O4 material evaluated at different current densities. Evidently, the anode presents superior rate capability for Li+ storage with averaged capacities of 603, 376, 175 and 92 mA·h/g at current densities of 100 mA/g, 200 mA/g, 500 mA/g and 1 A/g,respectively. After switching back to 100 mA/g, a reversible capacity of 340 mA·h/g can be recovered.
Figure 4 N2 adsorption/desorption isotherms (a) and pore size distribution (b) of MnO2 precursor and CoMn2O4 samples prepared after 10 min (CoMnO-10 min) and 1 h (CoMnO-1 h)
Figure 5 Electrochemical property of hierarchical spherical MnO2 material:
Figure 6 Electrochemical property of hierarchical spherical CoMn2O4 material:
The outstanding electrochemical properties of the MnO2 and CoMn2O4 materials can mainly be attributed to its unique structural characteristics:1) the hierarchical framework consisting of thin nanosheets building blocks endows a large electrode/electrolyte contact interface, facilitating better electrolyte permeation, diffusion, and shortened Li+ diffusion path lengths;2) the hierarchically porous network can buffer the repeated volume variation of the electrode during cycling; and 3) the coexistence of crystalline/ amorphous MnO2 domains leads to formation of abundant nanoscale coherent heterojunctions, featuring rapid Li+/electron transfer at heterointerfaces and enlarging Li+ storage at interfacial regions. The synergy of the above characteristics may enable the MnO2 electrode superior Li+ storage capability.
4 Conclusions
Hierarchically structured spherical phase- hybridized MnO2 and flowerlike single-crystal CoMn2O4 materials have been successfully synthesized by a one-pot or two-step wet-chemical routes at room temperature, respectively. The resultant materials are constructed by ultrathin nanosheets, presenting three-dimensional inter- penetrating porous networks featured with high surface areas (339 m2/g for MnO2 and 228 m2/g for CoMn2O4). Such unique structural characteristics endow the resultant materials with superior electrochemical properties. Preliminary results indicate that the MnO2 material exhibits high Li+ storage capability when evaluated as anode in Li-ion batteries. The present synthesis methodology may be extended for fabrication of a series of other transition metal oxides materials for promise applications in electrochemical energy conversion and storage.
References
[1] MASSE R C, UCHAKER E, CAO Guo-zhong. Beyond Li-ion: Electrode materials for sodium- and magnesium-ion batteries [J]. Science China-Materials, 2015, 58(9): 715–766. DOI: 10.1007/ s40843-015-0084-8.
[2] MASSE Robert C, LIU Chao-feng, LI Yan-wei, MAI Li-qiang, CAO Guo-zhong. Energy storage through intercalation reactions: Electrodes for rechargeable batteries [J]. National Science Review, 2017, 4(1): 26–53. DOI: 10.1093/nsr/nww093.
[3] LYU Fu-cong, SUN Zhi-fang, NAN Bo, YU Si-cen, CAO Lu-jie, YANG Ming-yang, LI Min-chan, WANG Wen-xi, WU Shao-fei, ZENG Shan-shan, LIU Hong-tao, LU Zhou-guang. Low-cost and novel Si-based gel for Li-ion batteries [J]. Acs Applied Materials & Interfaces, 2017, 9(12): 10699–10707. DOI: 10.1021/acsami.7b00460.
[4] LIU Jun, YU Li-tao, WU Chao, WEN Yu-ren, YIN Kui-bo, CHIANG Fu-kuo, HU Ren-zong, LIU Jiang-wen, SUN Li-tao, GU Lin, MAIER J, YU Yan, ZHU Min. New nanoconfined galvanic replacement synthesis of hollow Sb@C yolk-shell spheres constituting a stable anode for high-rate Li/Na-Ion batteries [J]. Nano Letters, 2017, 17(3): 2034–2042. DOI: 10.1021/acs.nanolett.7b00083.
[5] YANG Ying, ZHAO Xu, WANG Hong-en, LI Ma-lin, HAO Ce, JI Min, REN Su-zhen, CAO Guo-zhong. Phosphorized SnO2/graphene heterostructures for highly reversible lithium-ion storage with enhanced pseudocapacitance [J]. Journal of Materials Chemistry A, 2018, 6(8): 3479–3487. DOI: 10.1039/c7ta10435a.
[6] DONG Yu-cheng, YANG Shi-liu, ZHANG Zhen-yu, LEE Jong-min, ZAPIEN J A. Enhanced electrochemical performance of lithium ion batteries using Sb2S3 nanorods wrapped in graphene nanosheets as anode materials [J]. Nanoscale, 2018, 10(7): 3159–3165. DOI: 10.1039/ c7nr09441h.
[7] ZHAO Xu, SUI Jie-he, LI Fei, FANG Hai-tao, WANG Hong-en, LI Jiang-yu, CAI Wei, CAO Guo-zhong. Lamellar MoSe2 nanosheets embedded with MoO2 nanoparticles: novel hybrid nanostructures promoted excellent performances for lithium ion batteries [J]. Nanoscale, 2016, 8(41): 17902–17910. DOI: 10.1039/c6nr05584b.
[8] CAI Yi, WANG Hong-En, ZHAO Xu, HUANG Fei, WANG Chao, DENG Zhao, LI Yu, CAO Guo-zhong, SU Bao-lian. Walnut-like porous core/shell TiO2 with hybridized phases enabling fast and stable lithium storage [J]. ACS Applied Materials & Interfaces, 2017, 9(12): 10652–10663. DOI: 10.1021/ acsami.6b16498.
[9] HUANG Shao-zhuan, CAI Yi, JIN Jun, LIU Jing, LI Yu, WANG Hong-en, CHEN Li-hua, HASAN T, SU Bao-lian. Unique walnut-shaped porous MnO2/C nanospheres with enhanced reaction kinetics for lithium storage with high capacity and superior rate capability [J]. Journal of Materials Chemistry A, 2016, 4(11): 4264–4272. DOI: 10.1039/ c6ta00594b.
[10] HUANG Shao-zhuan, JIN Jun, CAI Yi, LI Yu, DENG Zhao, ZENG Jun-yang, LIU Jing, WANG Chao, HASAN T, SU Bao-lian. Three-dimensional (3D) bicontinuous hierarchically porous Mn2O3 single crystals for high performance lithium-ion batteries [J]. Scientific Reports, 2015, 5: 14686. DOI: 10.1038/srep14686.
[11] HUANG Shao-zhuan, CAI Yi, JIN Jun, LIU Jing, LI Yu, YU Yong, WANG Hong-en, CHEN Li-hua, SU Bao-lian. Hierarchical mesoporous urchin-like Mn3O4/carbon microspheres with highly enhanced lithium battery performance by in-situ carbonization of new lamellar manganese alkoxide (Mn-DEG) [J]. Nano Energy, 2015, 12: 833–844. DOI: 10.1016/j.nanoen.2015.01.040.
[12] LIU Chao-feng, ZHANG Chang-kun, SONG Huan-qiao, ZHANG Cui-ping, LIU Ya-guang, NAN Xi-hui, CAO Guo-zhong. Mesocrystal MnO cubes as anode for Li-ion capacitors [J]. Nano Energy, 2016, 22: 290–300. DOI: 10.1016/j.nanoen.2016.02.035.
[13] ZHANG Kai, HAN Xiao-peng, HU Zhe, ZHANG Xiao-long, TAO Zhan-liang, CHEN Jun. Nanostructured Mn-based oxides for electrochemical energy storage and conversion [J]. Chemical Society Reviews, 2015, 44(3): 699–728. DOI: 10.1039/c4cs00218k.
[14] LI Jing-fa, XIONG Sheng-lin, LI Xiao-wei, QIAN Yi-tai. A facile route to synthesize multiporous MnCo2O4 and CoMn2O4 spinel quasi-hollow spheres with improved lithium storage properties [J]. Nanoscale, 2013, 5(5): 2045–2054. DOI: 10.1039/c2nr33576j.
[15] SHI Jia-jia, LEI Kai-xiang, SUN Wei-yi, LI Fu-jun, CHENG Fang-yi, CHEN Jun. Synthesis of size-controlled CoMn2O4 quantum dots supported on carbon nanotubes for electrocatalytic oxygen reduction/evolution [J]. Nano Research, 2017, 10(11): 3836–3847. DOI: 10.1007/s12274- 017-1597-0.
[16] JIANG Cheng, YUAN Chen-pei, LI Pei-hang, WANG Heng-guo, LI Yan-hui, DUAN Qian. Nitrogen-doped porous graphene with surface decorated MnO2 nanowires as a high-performance anode material for lithium-ion batteries [J]. Journal of Materials Chemistry A, 2016, 4(19): 7251–7256. DOI: 10.1039/c5ta10711c.
[17] JAN S S, NURGUL S, SHI Xiao-qin, XIA Hui. Self- assembled microspheres formed from alpha-MnO2 nanotubes as an anode material for rechargeable lithium-ion batteries [J]. Journal of Nanoscience and Nanotechnology, 2015, 15(9): 7181–7185. DOI: 10.1166/jnn.2015.10552.
[18] WANG Hong-en, LU Zhou-guang, QIAN Dong, LI Yu-jie, ZHANG Wei. Single-crystal alpha-MnO2 nanorods: Synthesis and electrochemical properties [J]. Nanotechnology, 2007, 18(11): 115616. DOI: 10.1088/0957-4484/18/11/ 115616.
[19] LI Lei, RAJI A R O, TOUR J M. Graphene-Wrapped MnO2-graphene nanoribbons as anode materials for high-performance lithium ion batteries [J]. Advanced Materials, 2013, 25(43): 6298–6302. DOI: 10.1002/adma.201302915.
[20] XIA Hui, LAI Man-on, LU Li. Nanoflaky MnO2/carbon nanotube nanocomposites as anode materials for lithium-ion batteries [J]. Journal of Materials Chemistry, 2010, 20(33): 6896–6902. DOI: 10.1039/c0jm00759e.
[21] WANG Hong-en, ZHAO Xu, YIN Kai-li, LI Yu, CHEN Li-hua, YANG Xiao-yu, ZHANG Wen-jun, SU Bao-lian, CAO Guo-zhong. Superior pseudocapacitive lithium-ion storage in porous vanadium oxides@C heterostructure composite [J]. ACS Applied Materials & Interfaces, 2017, 9(50): 43665–43673. DOI: 10.1021/acsami.7b13658.
[22] SUN Ming-hui, HUANG Shao-zhuan, CHEN Li-hua, LI Yu, YANG Xiao-yu, YUAN Zhong-yong, SU Bao-lian. Applications of hierarchically structured porous materials from energy storage and conversion, catalysis, photocatalysis, adsorption, separation, and sensing to biomedicine [J]. Chemical Society Reviews, 2016, 45(12): 3479–3563. DOI: 10.1039/c6cs00135a.
[23] LI Jun-hua, JIANG Jian-bo, LIU Meng-qin, XU Zhi-feng, DENG Pei-hong, QIAN Dong, TONG Chao-ying, XIE Hao-bin, YANG Chun-ming. Facile synthesis of MnO2- embedded flower-like hierarchical porous carbon microspheres as an enhanced electrocatalyst for sensitive detection of caffeic acid [J]. Analytica Chimica Acta, 2017, 985: 155–165. DOI: 10.1016/j.aca.2017.07.002.
[24] HU Lin, ZHONG Hao, ZHENG Xin-rui, HUANG Yi-min, ZHANG Ping, CHEN Qian-wang. CoMn2O4 spinel hierarchical microspheres assembled with porous nanosheets as stable anodes for lithium-ion batteries [J]. Scientific Reports, 2012, 2: 986. DOI: 10.1038/srep00986.
[25] LI Chun, HAN Xiao-peng, CHENG Fan-gyi, HU Yu-xiang, CHEN Cheng-cheng, CHEN Jun. Phase and composition controllable synthesis of cobalt manganese spinel nanoparticles towards efficient oxygen electrocatalysis [J]. Nature Communications, 2015, 6: 7345. DOI: 10.1038/ncomms8345.
[26] KUANG Pan-yong, LIANG Min-hua, KONG Wan-yi, LIU Zhao-qing, GUO Yun-ping, WANG Hong-juan, LI Nan, SUA Yu-zhi, CHEN Shuang. Anion-assisted one-pot synthesis of 1D magnetic alpha- and beta-MnO2 nanostructures for recyclable water treatment application [J]. New Journal of Chemistry, 2015, 39(4): 2497–2505. DOI: 10.1039/ c4nj02196g.
[27] BIJELIC M, LIU Xiang, SUN Q, DJURISIC A B, XIE Mao-hai, NG A M C, SUCHOMSKI C, DJERDJ I, SKOKO Z, POPOVIC J. Long cycle life of CoMn2O4 lithium ion battery anodes with high crystallinity [J]. Journal of Materials Chemistry A, 2015, 3(28): 14759–14767. DOI: 10.1039/c5ta03570h.
[28] WANG Hong-en, QIAN Dong, LU Zhou-guang, LI Yong-kun, CHENG Rui-jing, LI Yu-jie. Facile synthesis and electrochemical properties of hierarchical MnO2 submicrospheres and LiMn2O4 microspheres [J]. Journal of Physics and Chemistry of Solids, 2007, 68(7): 1422–1427. DOI: 10.1016/j.jpcs.2007.02.041.
[29] CHOWDHURY A D, AGNIHOTRI N, SEN P, DE A. Conducting CoMn2O4-PEDOT nanocomposites as catalyst in oxygen reduction reaction [J]. Electrochimica Acta, 2014, 118: 81–87. DOI: 10.1016/j.electacta.2013.11.165.
[30] ZHU Han, YU Dan-ni, ZHANG Song-ge, CHEN Jia-wei, WU Wen-bo, WAN Meng, WANG Li-na, ZHANG Ming, DU Ming-liang. Morphology and structure engineering in nanofiber reactor: Tubular hierarchical integrated networks composed of dual phase octahedral CoMn2O4/Carbon nanofibers for water oxidation [J]. Small, 2017, 13(26): 1700468. DOI: 10.1002/smll.201700468.
[31] ZHANG Li-xin, HE Guo-feng, LEI Shi-wen, QI Gui-sheng, JIU Hong-fang, WANG Juan. Hierarchical hollow microflowers constructed from mesoporobs single crystalline CoMn2O4 nanosheets for high performance anode of lithium ion battery [J]. Journal of Power Sources, 2016, 326: 505–513. DOI: 10.1016/j.jpowsour.2016.07.021.
[32] ZHENG Jian-hua, HU Yan-dong, ZHANG Lei. Design and construction of a bifunctional magnetically recyclable 3D CoMn2O4/CF hybrid as an adsorptive photocatalyst for the effective removal of contaminants [J]. Physical Chemistry Chemical Physics, 2017, 19(36): 25044–25051. DOI: 10.1039/c7cp04395c.
[33] ZHANG Gen-qiang, LOU Xiong-wen. General synthesis of multi-shelled mixed metal oxide hollow spheres with superior lithium storage properties [J]. Angewandte Chemie-International Edition, 2014, 53(34): 9041–9044. DOI: 10.1002/anie.201404604.
[34] LI Zheng-quan, DING Yue, XIONG Yu-jie, YANG Qing, XIE Yi. One-step solution-based catalytic route to fabricate novel alpha-MnO2 hierarchical structures on a large scale [J]. Chemical Communications, 2005(7): 918–920. DOI: 10.1039/b414204g.
[35] CHEN Xiao-qiao, CAO Zhi-guang, XING Li-dan, LIAO You-hao, QIU Yong-cai, LI Wei-shan. Improved Li-storage performance with PEDOT-decorated MnO2 nanoboxes [J]. Nanoscale, 2017, 9(46): 18467–18473. DOI: 10.1039/ c7nr05654k.
[36] GUO Xian-wei, HAN Jiu-hui, ZHANG Ling, LIU Pan, HIRATA A, CHEN Lu-yang, FUJITA T, CHEN Ming-wei. A nanoporous metal recuperated MnO2 anode for lithium ion batteries [J]. Nanoscale, 2015, 7(37): 15111–15116. DOI: 10.1039/ c5nr05011a.
[37] REDDY A L M, SHAIJUMON M M, GOWDA S R, AJAYAN P M. Coaxial MnO2/Carbon nanotube array electrodes for high-performance lithium batteries [J]. Nano Letters, 2009, 9(3): 1002–1006. DOI: 10.1021/nl803081j.
[38] LIU Hong-dong, HU Zhong-li, RUAN Hai-bo, HU Rong, SU Yong-yao, ZHANG Lei, ZHANG Jin. Nanostructured MnO2 anode materials for advanced lithium ion batteries [J]. Journal of Materials Science-Materials in Electronics, 2016, 27(11): 11541–11547. DOI: 10.1007/s10854- 016-5284-9.
[39] LIU Hong-dong, HU Zhong-li, SU Yong-yao, RUAN Hai-bo, HU Rong, ZHANG Lei. MnO2 nanorods/3D-rGO composite as high performance anode materials for Li-ion batteries [J]. Applied Surface Science, 2017, 392: 777–784. DOI: 10.1016/j.apsusc.2016.09.104.
(Edited by FANG Jing-hua)
中文导读
等级结构锰氧化物的简易合成及其用于锂离子电池负极的研究
摘要:锰氧化物具有高的理论储锂容量同时原料丰富,有望用于高性能锂离子电池的负极材料。本文报道一种室温下的氧化还原沉淀反应合成具有等级球形二氧化锰的方法。该方法简便有效,同时得到的材料具有无定形和晶畴共存的结构特点。以其为前驱物,进一步制备了单晶纳米片组装的花形尖晶石型锰酸钴。该温和的合成方法不同于传统的水热或固相合成方法,能够避免颗粒间因团聚引起的活性表面的损失。所得到的二氧化锰和锰酸锂均具有优异的电化学储锂性能,有望用于锂离子电池和其它储能领域。
关键词:氧化锰;纳米结构;阳极材料;锂离子电池;电化学
Foundation item: Project(JCYJ20170817110251498) supported by the Basic Research Project of the Science and Technology Innovation Commission of Shenzhen, China; Project(2016TQ03C919) supported by the Guangdong Special Support for the Science and Technology Leading Young Scientist, China; Project(21603094) supported by the National Natural Science Foundation of China
Received date: 2018-10-19; Accepted date: 2019-02-21
Corresponding authors: CHENG Hua, PhD, Associate Professor; Tel: +86-755-88018915; E-mail: chengh@sustc.edu.cn; ORCID: 0000-000209990-7504; WANG Hong-en, PhD, Associate Professor; Tel: +86-27-87879468; E-mail:hongenwang@whut.edu.cn; ORCID: 0000-0002-6859-5683
Abstract: Developing high-performance lithium ion batteries (LIBs) using manganese oxides as anodes is attractive due to their high theoretical capacity and abundant resources. Herein, we report a facile synthesis of hierarchical spherical MnO2 containing coherent amorphous/crystalline domained by a simple yet effective redox precipitation reaction at room temperature. Further, flower-like CoMn2O4 constructed by single-crystalline spinel nanosheets has been fabricated using MnO2 as precursor. This mild methodology avoids undesired particle aggregation and loss of active surface area in conventional hydrothermal or solid-state processes. Moreover, both MnO2 and CoMn2O4 nanosheets manifest superior lithium-ion storage properties, rendering them promising applications in LIBs and other energy-related fields.
- Facile synthesis of hierarchically structured manganese oxides as anode for lithium-ion batteries
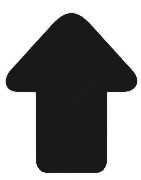