J. Cent. South Univ. Technol. (2007)04-0496-04
DOI: 10.1007/s11771-007-0096-y
Numerical simulation of temperature and velocity fields in plasma spray
FAN Qun-bo(范群波), WANG Lu(王 鲁), WANG Fu-chi(王富耻)
(School of Materials Science and Engineering, Beijing Institute of Technology, Beijing 100081, China)
_______________________________________________________________
Abstract:
Based on the turbulence jet model, with respect to Ar-He mixture plasma gas injecting to ambient atmosphere, the temperature filed and velocity field under typical working conditions were investigated. Given the conditions of I=900 A, FAr= 1.98 m3/h, FHe=0.85 m3/h, it is found that both the temperature and the velocity undergo a plateau region near the nozzle exit (0-10 mm) at the very first stage, then decrease abruptly from initial 13 543 K and 778.2 m/s to 4 000 K and 260.0 m/s, and finally decrease slowly again. Meanwhile, the radial temperature and radial velocity change relatively slow. The inner mechanism for such phenomena is due to the complex violent interaction between the high-temperature and high-velocity turbulent plasma jet and the ambient atmosphere. Compared with traditional methods, the initial working conditions can be directly related to the temperature and velocity fields of the plasma jet by deriving basic boundary conditions.
Key words:
plasma spray; plasma jet; temperature field; velocity field;
_______________________________________________________________
1 Introduction
In plasma spray, the distributions of temperature and velocity of the plasma jet, namely temperature field and velocity field, would influence the molten status and acceleration of the sprayed particles greatly, thus determining the final coating’s quality [1]. However, it is usually difficult to measure all these key parameters of the plasma jet directly by employing existent apparatuses due to its extremely high temperature (>104 K at local positions) and relatively high velocity (up to 700 m/s). With the fast development of hardware and software of computers in recent years, numerical simulation method has become one effective substituted way. ECKERT et al[2] have investigated the heat conduction of the plasma jet, which formed the initial foundation of this research, but most of the early work is limited to studying simply the laminar flow[3]. In practical working conditions, however, the plasma jet often behaviors as a turbulent flow, due to its large size and flow rates, as well as large powers. Though there is still no perfect turbulent-flow model for plasma jet up to the present time[4], people often follow the methods used in describing non-ionic gases, such as turbulent kinetic energy-dissipation rate equations[5]. Therefore in this study, through inputting the initial and boundary conditions at the nozzle exit, the temperature field and velocity field of Ar-He plasma jet flowing into the atmosphere under typical operation conditions were simulated using the professional CFD software Fluent 6.0.
2 Mathematical modelsThe model is based on the following assumptions:
1) The plasma is regarded as a continuous media[4] and in local thermodynamic equilibrium[6-7];
2) The plasma gas is composed of two species: primary gas argon (Ar) and secondary gas helium (He);
3) The plasma gas is optic thin, so the energy loss by radiation is negligible;
4) The plasma gas behaves as a Newtonian fluid;
5) During a certain period, the plasma jet flow is in a steady-state system[6-8].
2.1 Basic governing equationsThe plasma jet satisfies three governing equations: mass conservation equation, momentum conservation equation, and energy conservation equation.
2.1.1 Conservation of mass
Based on the assumption that there is no mass added to the continuous plasma gas, the rate of change of the fluid mass in control volume is equal to the overall fluid mass flux into the volume, which may be expressed as
(1)
where ρ is the local density of the plasma gas; t is the time; v is the gas velocity vector; fi is the net production rate of species i, and ∑fi=1.
2.1.2 Conservation of momentum
According to the law of momentum conservation, the rate of change of momentum of fluid within any control volume is equal to the total body and surface forces produced by external means and doing work on the fluid volume. Neglecting external body forces, the momentum conservation equation [9] is given by
(2)
where P is the pressure and τ is the stress tensor.
Eqn.(2) is applicable for all species, including the mixture gas.
2.1.3 Conservation of energy
Based on the assumptions mentioned before that the energy loss by radiation and chemical reactions are negligible, the rate of change of fluid enthalpy within a control volume is equal to the rate at which the heat is conducted into the fluid, and the rate at which body and surface forces acting on the fluid. The conservation of energy can therefore be given by
(3)
where κ is thermal conductivity; h is the specific enthalpy of the mixture gas; Ф is the rate of energy loss by viscous frictions, which is turned into the thermal form; T is the temperature.
2.2 k-ε equationsThe thermal plasma jet is a highly turbulent flow; the modern approaches have formed a strong attachment to the methodology of the discipline of turbulence modeling work of ordinary turbulent flow. However, there are many special features of turbulent thermal plasma, which make the modeling work more difficult.
To accurately describe the turbulent characteristics of the free plasma jet, the famous k-ε model[9-11] is introduced, which includes two differential equations for the turbulent kinetic energy k and for its dissipation rate ε. The equations take the following form:
(4)
(5)
where vx and vr are the axial and radial velocity components, respectively; ρ is the density of the plasma jet and μ is the viscosity in laminar flow situations; μt is the turbulent viscosity and can be obtained by μt=cμρk2/ε; k is kinetic energy; ε is dissipation rate. c1, c2, cμ, σk, σε are constants with the values of 1.44, 1.92, 0.09, 1.0 and 1.3, respectively.
3 Geometry and boundary conditionsFig.1 shows the geometry and computational domain. A rectangular mesh is used (the axial direction is set to x-coordinate, and the radial direction is set to r-coordinate), subdivided in a non-uniform 80×50 grid. In the radial direction, the grid is more refined near the central axis and coarser towards the outer environment. As shown in Fig.1, the nozzle exit is located at line AB, with the radius of 4 mm, which is also the inlet of the plasma field, and the coordinate origin is just at the center of the nozzle exit.
Fig.1 Calculation domain
At the inlet of the plasma jet, the initial kinetic and thermodynamics boundary conditions shall be given before calculation, including the initial temperature T0, the initial velocity v0, the initial kinetic energy k0, and the initial dissipation rate ε0. Table 1 shows some of the basic parameters under typical operation conditions, which are obtained through the method of energy conservation in the plasma gun[12].
Table 1 Initial boundary conditions under typical operation condition
The values of k0 and ε0 can be given by experiments or simply the empirical estimation. Since the jet near the center of the plasma gun is usually a laminar flow before it transfers to a turbulent flow[6], we define that both k0 and ε0 are equal to 0, while at the outer boundaries, such as BC, CD, DE, EF and FA, the turbulence is specified as 10%, and the corresponding turbulent characteristic length is 1/4 of the nozzle exit, namely 2 mm.
The temperature, pressure and the mass fractions of species at BC, CD, DE, EF and FA are specified to be 300 K, the atmospheric pressure and zero, respectively.
4 Results and discussion 4.1 Temperature field under typical operation conditionsFig.2 shows the temperature field of the plasma jet under typical operation conditions (I=900 A, FAr=1.98 m3/h, FHe=0.85 m3/h). Fig.2(a) shows the temperature contours, with the temperature interval of about 800 K, Fig.2(b) shows the temperature distribution at different radial positions (r≥0) along the axis. It can be seen from Fig.2 that when high-temperature plasma jet leaves the nozzle exit, the temperature decreases rapidly, in both the axial and radial directions. Given the fixed initial conditions, the plasma temperature field is in axial symmetry centered in the axis x.
It can be seen from Fig.2 that when the high-temperature plasma jet diffuses into the atmosphere, the temperature goes through a plateau near the nozzle exit (0-10 mm) with the value of 13 534 K at the very first stage, then decreases rapidly to about 4 000 K. The temperature contour density in Fig.2 reflects the temperature changing rate: denser contours correspond to relatively higher changing rate. Fig.2(a) shows that the temperature in the axial direction changes slowly firstly, then sharply, and finally slowly again, which can also be seen from the Temperature-x curve at the position of r=0. Fig.2(b) shows that given the same axial position, temperature T in the radial direction also changes in three stages: slowly firstly, then sharply, and finally slowly again. With the increase of the axial position, the changes will become more and more slowly. In the outer region of the plasma jet, the temperature decreases to less than 1 500 K. When the high-temperature jet diffuses into the room temperature atmosphere, not only materials interchange but also heat interchange will happen. At the outer interface of the turbulent plasma jet, the temperature decreases nearly to 1 500 K due to the atmosphere’s cooling effects.
Fig.2 Temperature field under typical working conditions
(a) Temperature contours(K); (b) Temperature at different radial positions along axis
4.2 Velocity field under typical operation conditionsFig.3 illustrates the velocity field of the plasma jet under typical operation conditions (I=900 A, FAr=1.98 m3/h, FHe=0.85 m3/h). Fig.3(a) shows the velocity contours, with the velocity interval of about 50 m/s, Fig.3(b) shows the velocity distribution at different radial positions (r≥0) along the axis. It can be seen from Fig.3 that when high-velocity leaves the nozzle exit, the velocity decreases rapidly, in both the axial and radial directions. Given the fixed initial conditions, the plasma velocity field is in axial symmetry centered in the axis x.
Fig.3 Velocity field under typical working conditions
(a) Velocity contours (m/s)(b) Velocity at different radial positions along axis
It can be seen from Fig.3 that when the high-velocity plasma jet diffuses into the atmosphere, the velocity goes through a plateau near the nozzle exit (0-10 mm) with the value of 778.2 m/s at the very first stage, then decreases rapidly to about 260 m/s. Similarly, the velocity contour density in Fig.3 also reflects the velocity changing rate: denser contours correspond to relatively higher changing rate. Fig.3(a) shows that the velocity in the axial direction changes slowly firstly, then sharply, and finally slowly again by comparing the contour density along the axis x, which can also be seen from the velocity-x curve at the position of r=0. Fig.3(b) shows that, velocity at the same axial position in the radial direction also changes in three stages: slowly firstly, then sharply, and finally slowly again. With the increase of the axial position, the changes will become more and more slowly. In the outer region of the plasma jet, the velocity decreases to less than 45 m/s due to the entrainment effect of the atmosphere on the plasma jet. It might be noted that the axial velocities in some outer regions are negative, indicating that the velocities are in direction x, which is just caused by the entrainment effect. Such phenomena was also reported in Ref.[13]. When the plasma jet is injected into the atmosphere, the irregular movement of the particles, especially transverse fluctuation velocities will introduce the momentum interaction between the jet and the ambient medium, thus increasing the mass of the fluid, expanding its width, and gradually immersing into the plasma’s center.
As can be seen in both Figs.2 and 3, the initial plateau is just the plasma core, indicating that the atmosphere has not penetrated. Because of the entrainment of the atmosphere, the temperature and velocity of the plasma jet decrease rapidly. But in the outer region far from the plasma core, this effect is weakened greatly.
5 Conclusions1) The k-ε equations are combined with basic governing equations to calculate the turbulent behaviors of the plasma jet. The results agree well with that in related literatures.
2) Comparing with works reported in other literatures, this paper successfully relates the initial working conditions to the temperature fields and velocity fields of the plasma jet, instead of presenting experiential boundary conditions.
3) Under typical working conditions, the temper- ature and velocity decrease both axially and radially, going through three stages: slowly firstly, then sharply, and finally slowly again.
4) The initial plateau corresponds to the plasma core, with the entrainment of the atmosphere, the temperature and the velocity of the plasma jet decrease rapidly, but at the outer regions, this effect is weakened.
References[1] FINCKE J R, SWANK W D, BEWLE R L, et al. Diagnostics and control in the thermal spray process [J]. Surface and Coatings Technology, 2001, 146-147: 537-543
[2] ECKER E R G, PFENDER E. Advances in Plasma Heat Transfer[M]. New York: Academic Press, 1967.
[3] LEE Yung-cheng. Modeling Work in Thermal Plasma Process[D]. Minneapolis: University of Minnesota, 1984.
[4] CHEN Xi. Heat Transfer and Flow of High-temperature Ionic Gas[M]. Beijing: Science Press, 1993. (in Chinese)
[5] CHANG C H, RAMSHAW J D. Modeling of nonequilibrium effects in a high-velocity nitrogen-hydrogen plasma jet[J]. Plasma Chemistry and Plasma Processing, 1996, 16(s1): 5-17.
[6] DUSSOUBS B, FAUCHAIS P, VARDELLE A, et al. Computational analysis of a three-dimensional plasma spray jet[C]// XXIII International Conference on Phenomena in Ionized Gases, Toulouse: Centre de Phys Plasmas et leurs Applications de Toulouse, 1997, 2: 158-159.
[7] NISHIYAMA H, KUZUHARA M, SOLONENKO O P, et al. Numerical modeling of an impinging dusted plasma jet controlled by a magnetic field in a low pressure[C]// Proceeding of the International Thermal Spray Conference. Nice: ASM International, 1998: 451-456.
[8] DUSSOUBS B, VARDELLE A, MARIAUX G, et al. Modeling of plasma spraying of two powders[J]. Journal of Thermal Spray Technology, 2001, 10(1): 105-110.
[9] CHEN Ke-fa, FAN Jian-ren. Theory and Calculation of Engineering Gas-solid Multi-phase Fluid[M]. Hangzhou: Zhejiang University Press, 1990. (in Chinese)
[10] XIE Hong. Theory and Experimental Study of Plasma Jet with Particles[D]. Beijing: Tsinghua University, 1988. (in Chinese)
[11] SU Bin-zhi. Flow and Heat Transfer of Thermal Plasma[D]. Beijing: Tsinghua University, 1988. (in Chinese)
[12] FAN Qun-bo, WANG Lu, WANG Fu-chi. Numerical simulation of basic parameters in plasma spray[J]. Journal of Beijing Institute of Technology, 2004, 13(1): 80-84.
[13] SCHLICHTING H, GERSTEN K, KRAUSE E, et al. Boundary-Layer Theory[M]. New York: Springer-Verlag, 2000.
_________________________
Foundation item: Project (9140A12020306BQ0117) supported by the Commission of Science Technology and Industry for National Defense; Project ( 1040012040101) supported by the Excellent Young Teacher Foundation of Beijing Institute of Technology
Received date: 2006-08-24; Accepted date: 2006-09-27
Corresponding author: FAN Qun-bo, Lecturer, PhD; Tel: +86-10-89108185; E-mail: fanqunbo@163.com
(Edited by ZHAO Jun)
- Numerical simulation of temperature and velocity fields in plasma spray
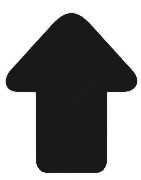